Color blindness, also known as color vision deficiency (CVD) or Daltonism, is a visual impairment characterized by the inability or reduced ability to perceive certain colors.
Color vision relies on specialized photoreceptor cells known as cone cells, located within the retina of the eye. These cone cells contain photopigments that respond to different wavelengths of light, allowing the perception of various colors.
Cones vary in number from 6 to 7 million, are responsible for the visual acuity of the human eye, the ability of the eye to resolve and to pick up the minor details on an object, and for distinguishing colors. They are concentrated in the small central part of the retina known as the Fovea Centralis, measuring 0.3 millimetres across and devoid of rods.
There are three primary types of cone cells, each containing a specific type of opsin protein that determines its sensitivity to different wavelengths of light:
- Long-wavelength sensitive cones (L cones or Red cones) contain the opsin protein known as long-wavelength opsin (L opsin) or Red pigment. These cones are primarily responsible for perceiving red colors and are most sensitive to long wavelengths of light.
- Middle-wavelength sensitive cones (M cones or Green cones) contain the opsin protein called middle-wavelength opsin (M opsin) or Green pigment. M cones are specialized in perceiving green colors and are most sensitive to middle wavelengths of light.
- Short-wavelength sensitive cones (S cones or Blue cones) contain the opsin protein referred to as short-wavelength opsin (S opsin) or Blue pigment. These cones are dedicated to detecting blue colors and are most sensitive to short wavelengths of light.
The functionality of all three cone types is needed to provide our ability to have a normal colors vision. Red and green cones make up 95% of the cones in the fovea, while blue cones account for only 5%.
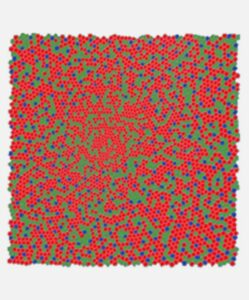
Retina (Top View) – Cone rich Fovea Centralis
The brain processes and integrates signals from the different types of cone cells to create a comprehensive spectrum of color perception.
In people with color blindness, genetic mutations or changes in the genes responsible for encoding these opsin proteins can cause abnormalities in cone cell function. This can lead to difficulties in discriminating between colors or perceiving colors differently compared to those with typical color vision, depending on which specific opsin is affected.
Prevalence and Causes
Color blindness can have different etiologies, including genetic and environmental factors. The predominant cause is typically inherited genetic mutations affecting the genes accountable for generating photopigments within cone cells.
Molecular genetics of human color vision studies the genes encoding blue, green, and red pigments [7,8].
The gene responsible for the formation of the blue photopigment is on chromosome 7 and tritanopia, that is the form of dichromacy due to a lack of functionality of blue cone cells in the retina, has an autosomal dominant inheritance. This means that only one of the two 7-chromosomes needs to carry a mutation in order to lack the functionality of S-cones.
Genes responsible for the L (long-wavelength sensitive) and M (middle-wavelength sensitive) photopigments are located adjacently on the X chromosome. Notably then, red and green color blindness is more commonly expressed in males (XY) than females (XX). Since males (XY) have only one X chromosome, they are more likely to be colorblind if there is a defect in their genes. Females (XX), on the other hand, have two X chromosomes, so even if one of the two X chromosomes carries a defective gene, for the X-inactivation process the not defective gene on the other X chromosome is going to be expressed.
Types of Color Blindness
Color blindness can be classified into various types depending on the specific cones and opsins affected. Typically, humans are trichromats, meaning they possess all three types of functional cone cells (L, M, and S) in their retinas, enabling them to perceive a broad spectrum of colors.
Normal Color Vision
Protanopia
Deuteranopia
Tritanopia
BCM
Achromatopsia
Dichromacy is characterized by individuals possessing only two functioning types of cone cells in their retinas, rather than the typical three. There are three main types of dichromacy, each characterized by the inability to distinguish specific pairs of colors:
- Protanopia: Individuals with protanopia lack functionality of red cone cells in their retinas, leading to difficulty discerning between red and green colors. Protanopia is due to a lack of the red photopigment caused by a mutation on the X-Chromosome opsin genes. The pattern of inheritance is X-linked recessive.
- Deuteranopia: Deuteranopia arises from a lack of functionality green cone cells in the retina, resulting in difficulty distinguishing between red and green colors. Deuteranopia is due to a lack of the green photopigment caused by a mutation on the X-Chromosome opsin genes. The pattern of inheritance is X-linked recessive.
- Tritanopia: This form of dichromacy is due to a lack of functionality of blue cone cells in the retina, resulting in difficulty discerning between blue and yellow colors. It occurs much less frequently compared to protanopia and deuteranopia. Tritanopia is due to a lack of the blue photopigment caused by mutations on gene S-opsin on chromosome 7. The pattern of inheritance is autosomal dominant.
Picture from http://www.neitzvision.com/
Anomalous trichromacy is the most common form of color vision deficiency where individuals have three types of cone cells, but one or more of these cone types exhibit abnormal sensitivity to certain wavelengths of light. As a result, individuals with anomalous trichromacy encounter difficulty distinguishing between specific colors, particularly shades of red and green. While dichromacy and anomalous trichromacy represent variations in color perception, individuals affected by them generally maintain relatively normal visual acuity, usually assessed using the 20/20 scale.
Finally, there are monochromacies, that are inherited retinal diseases. Symptoms are severe visual impairment with loss of visual acuity, nystagmus, photophobia and scarce or null color discrimination.
Blue Cone Monochromacy occurs when a genetic mutation causes the lack of both red and green photopigments. In this condition, perception of colors is severely impaired, and daylight vision is due to S-cones and rods. Visual acuity ranges between 20/60 and 20/200. Individuals affected by Blue Cone Monochromacy show intolerance to light and photophobia, very often show myopia and nystagmus. Nystagmus develops during the first few weeks after birth and in almost half of individuals it persists into adulthood. Blue Cone Monochromacy is due to mutations on the OPN1LW and OPN1MW genes on the X Chromosome and is an X-linked disease.
Rod monochromacy, also called Achromatopsia, represents the most severe form of color blindness. In this condition, none of the cone cells are functional. Achromatopsia is a rare genetic disease [11] characterized by reduced visual acuity, pendular nystagmus, increased sensitivity to light (photophobia), a small central scotoma, eccentric fixation, and reduced or complete loss of color discrimination. All individuals with achromatopsia have impaired color discrimination. Hyperopia is common in achromatopsia. Nystagmus develops during the first few weeks after birth followed by increased sensitivity to bright light. Best visual acuity varies with severity of the disease; it is 20/200 or less in complete achromatopsia and may be as high as 20/80 in incomplete achromatopsia. Visual acuity is usually stable over time; both nystagmus and sensitivity to bright light may improve slightly. Achromatopsia is inherited in an autosomal recessive manner. The mutations that cause achromatopsia result in disruption of the phototransduction signaling cascade. So, while the photopigments may be present and can absorb light, the cones cannot signal this to the brain and are thus all non-functional (red, green and blue). Identification of biallelic pathogenic variants in ATF6, CNGA3, CNGB3, GNAT2, PDE6C, or PDE6H confirms the clinical diagnosis.
What happens in people with BCM?
People with Blue Cone Monochromacy (BCM) lack the middle-wave (green) and long-wave (red) opsins, limiting their retina’s ability to capture light solely to the rods and blue cones. In Clinical Studies scientists obtained that L/M stimuli on a standard background light are seen by the rod system; shorter wavelength stimuli were seen either by the S- (blue) cone system or the rod system, depending on the retinal location and specific patient. The standard background light is the luminance of a white paper in typical indoor lighting, and it was somewhat unexpected to observe retained night vision under day vision conditions in BCM. The same happens with white light, which is composed of a mixture of red, green and blue light.
With only the blue cones and rods functioning, BCM patients have diminished detail vision (poor acuity) and difficulty, or the inability, to discern colors effectively. Typically, individuals with BCM can read only the top 1 to 4 lines of an eye chart, with their best corrected visual acuity ranging between 20/200 and 20/60.
Color vision in individual with BCM is severely impaired. Below a picture, created by a member of the BCM Families Foundation community, Dean Monthei, shows color vision difference between an individual with normal vision and an individual affected by BCM:
Picture courtesy of Dean Monthei
Demo glasses to simulate Blue Cone Monochromacy color vision by blocking red light and to simulate poor visual acuity have been developed.
The below bar shows a full spectrum of colors ranging from blue, then green and ending on red. The bottom half is normal color vision and the top half is what an individual with BCM sees.
Picture is a courtesy of Dean Monthei
For several members of the BCM Families Foundation community the top and bottom half of the color bars look identical.
The following picture show on the left a contrast white over yellow, that normal visual individuals cannot see very well, while individuals with BCM can easily read. On the right there is instead a contrast white over light blue that individuals with BCM cannot read. Results may vary with individual and/or the display being used.
Treatment
There is no known cure for color vision deficiency at the present moment. However, a groundbreaking study led by vision scientists Jay Neitz and Maureen Neitz at the University of Washington made a significant stride in color vision deficiency research. By targeting red-green color blindness and utilizing squirrel monkeys as models, the researchers introduced a corrective gene into the retinas of color blind individuals. This pioneering approach resulted in the restoration of red-green color vision in previously color blind squirrel monkeys. Despite this promising advancement, additional research is needed to evaluate the long-term safety, efficacy, and suitability of gene therapy for treating color vision deficiency in humans before it can be translated into clinical applications.
A squirrel monkeys, Dalton, who was treated for red-green color blindness
http://www.neitzvision.com/test/research/gene-therapy/
External Resources
- What Is Color Blindness? – American Academy of Ophthalmology. https://www.aao.org/eye-health/diseases/what-is-color-blindness
- Color Blindness | National Eye Institute. https://www.nei.nih.gov/learn-about-eye-health/eye-conditions-and-diseases/color-blindness
- Types of Colour Blindness – Colour Blind Awareness. https://www.colourblindawareness.org/colour-blindness/types-of-colour-blindness/
- The Basics – Neitz Vision. (n.d.). The Basics – Neitz Vision. http://www.neitzvision.com/test/the-basics/
- What Is Color Blindness? (2023, September 1). WebMD. https://www.webmd.com/eye-health/color-blindness
References:
- Deeb S S, (2005). “The molecular basis of variation in human color vision”. Clin. Genet. 67 (5): 369-377. DOI: 10.1111/j.1399-0004.2004.00343.x
- Mancuso K, Hauswirth W W, Li Q, Connor T B, Kuchenbecker J A, Mauck M C, Neitz J, Neitz M, (2009) “Gene therapy for red-green colour blindness in adult primates”. Nature (2009) 461:784-787. PMID: 19759534.
- Mancuso K, Mauck M C, Kuchenbecker J A, Neitz M, and Neitz J, (2010) “A Multi-Stage Color Model Revisited: Implications for a Gene Therapy Cure for Red-Green Colorblindness’2 (2010) R.E. Anderson et al. (eds.), Retinal Degenerative Diseases, Advances in Experimental Medicine and Biology 664. PMID: 20238067.
- Mascio A A, Roman A J, Cideciyan A V, Sheplock R, Wu V, Garafalo A V, Sumaroka A, Pirkle S, Kohl S, Wissinger B, Jacobson S G and Barbur J L, (2023) “Color vision in blue cone monochromacy: outcome measures for a clinical trial” Transl Vis Sci Techno 2023 Jan 3;12(1):25 DOI: 10.1167/tvst.12.1.25
- Nathans, J (1987). “Molecular biology of visual pigments”. Annu. Rev. Neurosci 10: 163-194. PMID 3551758.
- Nathans, J (1999). “The evolution and physiology of human color vision: insights from molecular genetic studies of visual pigments”. 24 (2): 299-312. PMID 10571225
- Nathans J, Piantanida T P, Eddy R L, Shows T B, Hogness D S, (1986). “Molecular genetics of inherited variation in human color vision”. Science 232 (4747): 203-210. PMID 3485310.
- Nathans J, Thomas D, Hogness D S, (1986). “Molecular genetics of human color vision: the genes encoding blue, green, and red pigments”. Science 232 (4747): 193-202. PMID 2937147
- Neitz J, Neitz M, (2011). “The genetics of normal and defective color vision”. Vision Res. 51 (7): 633–651. doi:1016/j.visres.2010.12.002.
- Neitz M, (2000). “Molecular Genetics of Color Vision and Color Vision Defects”. Archives of Ophthalmology. 118 (5): 691–700. doi:1001/archopht.118.5.691.
- Kohl S, Jägle H, Wissinger B, et al. Achromatopsia. 2004 Jun 24 [Updated 2018 Sep 20]. In: Adam MP, Feldman J, Mirzaa GM, et al., editors. GeneReviews® [Internet]. Seattle (WA): University of Washington, Seattle; 1993-2024. Available from: https://www.ncbi.nlm.nih.gov/books/NBK1418/